The existence of the pion π was established in 1947 by observations of the characteristic π→ μ+ν decay in nuclear emulsions. It was shown that in this process the μ are emitted with nearly constant velocity, with a mean energy of ejection of 4,12 MeV. The constant velocity of emission of the μ proves that the parent π particle is commonly reduced to a very low velocity in the emulsion before decaying, and that it transforms into a μ and one neutral particle (ν, neutrino) only. The mass of the pion was found to be 273 me (139,5 MeV/c²) and the muon 107 me (105,7 MeV/c²).
Left picture (1947) : First observation of the decay of a π. The parent particle π reached the end of its range at the point A and the secondary μ was ejected nearly backwards along the line of motion of the π. The variation in the scattering and grain density along the tracks made it evident that both particles were much less massive than a proton, and allowed their directions of motion to be established without ambiguity. The range of μ could not be identified as the particle left the emulsion.
Right picture (1947) : This second example of the decay of a π was found shortly after the first. Although the track of the parent particle is short, it give unambiguous evidence for the direction of motion of the particle and for its low mass. The secondary μ came to rest in the emulsion, its range being 610 μm which correspond to an initial kinetic energy of 4,1 MeV.
The π decay mode are :
The complete π+/- decay is pictured here. The end of range of π– in nuclear emulsion is pictured here. The pions (+ or -) can decay in flight or when they are brought to rest (they have no kinetic energy left). Note : the decay in flight of pion is a rare process. In 1953 Fry found 12 examples of decay in flight among 11800 π+ arrested in emulsion.
For the π– particles, observing the decay (at rest) is rare because this particle interact strongly with nucleus (see below). The decay of π– in emulsion has been reported by Fry (1954) who found the frequency of the process to be about one in 1000 of the entering π– .
π– capture by nucleus
Similar to the process of μ– capture by nuclei, π– particles can also be captured by nuclei once they lose all their kinetic energy. But as the pion is a strongly interacting particle with nuclei (about 1013 times more than muon), the disintegration of nuclei following the pion capture is more ‘violent’ than in muon capture (more ejection of alpha particle for example). When a π– is captured by a nucleus, it interacts with two or more nucleons, and a large fraction of the energy corresponding to the rest-mass of the pion can appear in the resulting disintegration.
Light or heavy element have equal probability to capture a pion. Three methods permit to determinate if it was a light or heavy element that captured a pion and disintegrated :
1.Similar to the observations with negative muon, that when Auger electrons are emitted in the disintegration, and if their range is observable, the disintegration can be associated by the capture of a muon or a pion by a heavy element.
2. Another method of distinguishing the disintegrations of light and heavy elements by pion capture was made with the ‘sandwich C2 emulsion’ in which thin layers of pure gelatine (light elements) were inter-leaved with those of a normal C2 emulsion (made of gelatine + the high elements Ag, Br). Disintegrations produced by the nuclear capture of a pion arrested in the gelatine can be distinguished by the fact that the origin of disintegration, located in photographically insensitive material, is not recorded. Such events therefore certainly correspond to the disintegration of a light element. See these examples of plates.
3. It is assumed that an α particle emitted with an energy less than that corresponding to the height of the potential barrier of silver or bromine (< 25 MeV) is only likely to appear trough the disintegration of a light nucleus (carbon, nitrogen or oxygen).
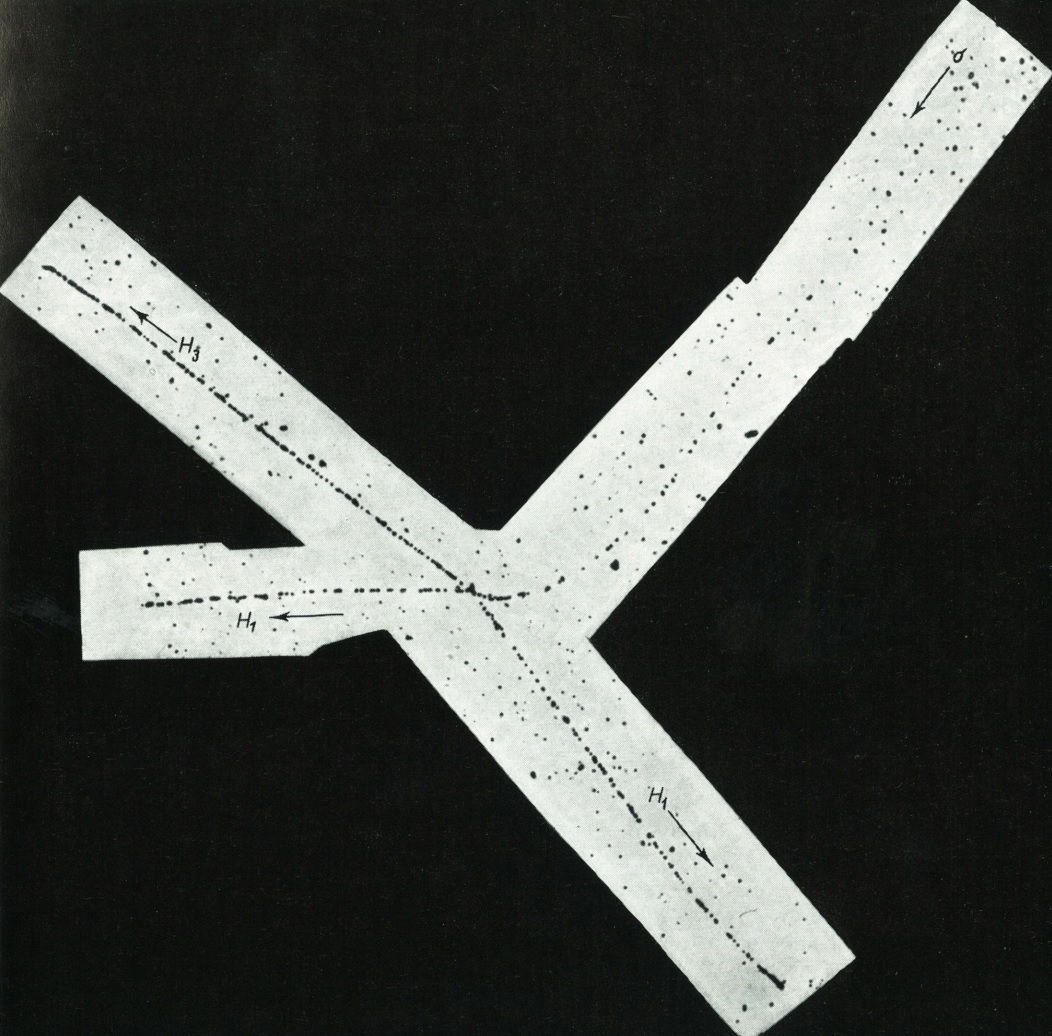
First observation of a disintegration by a negative pion, 1947. H1 are proton, H3 triton. σ is a π– particle.
Left picture : The change in grain density as the pion approaches the end of its range, and the increased scattering are clearly displayed. These features provided the decisive evidence that the particle had indeed approached the centre of disintegration and had no receded from it ; and that the particle, which must have produced the disintegration, was moving very slowly when it interacted with the nucleus. If followed that the energy of disintegration was provided by the disappearance of rest-mass of the incident particle, a process which had not previously been observed (in 1947).
The good discrimination of this early emulsion is well displayed, the differences in the rate of change of grain density along the tracks of the pion and of the secondary hydrogen nuclei being clearly apparent. The attribution of one of the tracks to a triton depends upon a comparison of the rates of change of grain density along the tracks.
.
.
.
.
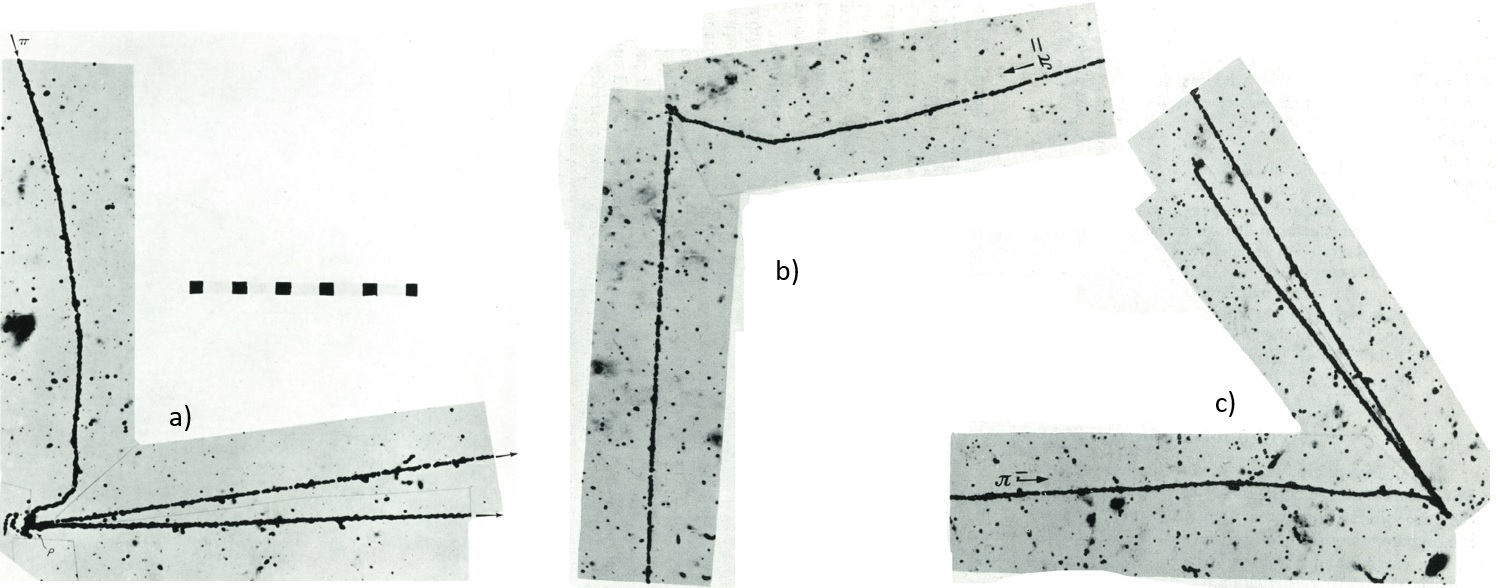
Disintegration of heavy elements, silver or bromine by the nuclear capture of π– particles. As an evidence, note the presence of Auger electrons in a) and b). This is not a fast nuclear collision by a pion as the heavy scattering of the pion nears the atom show that it’s kinetic energy is very low.
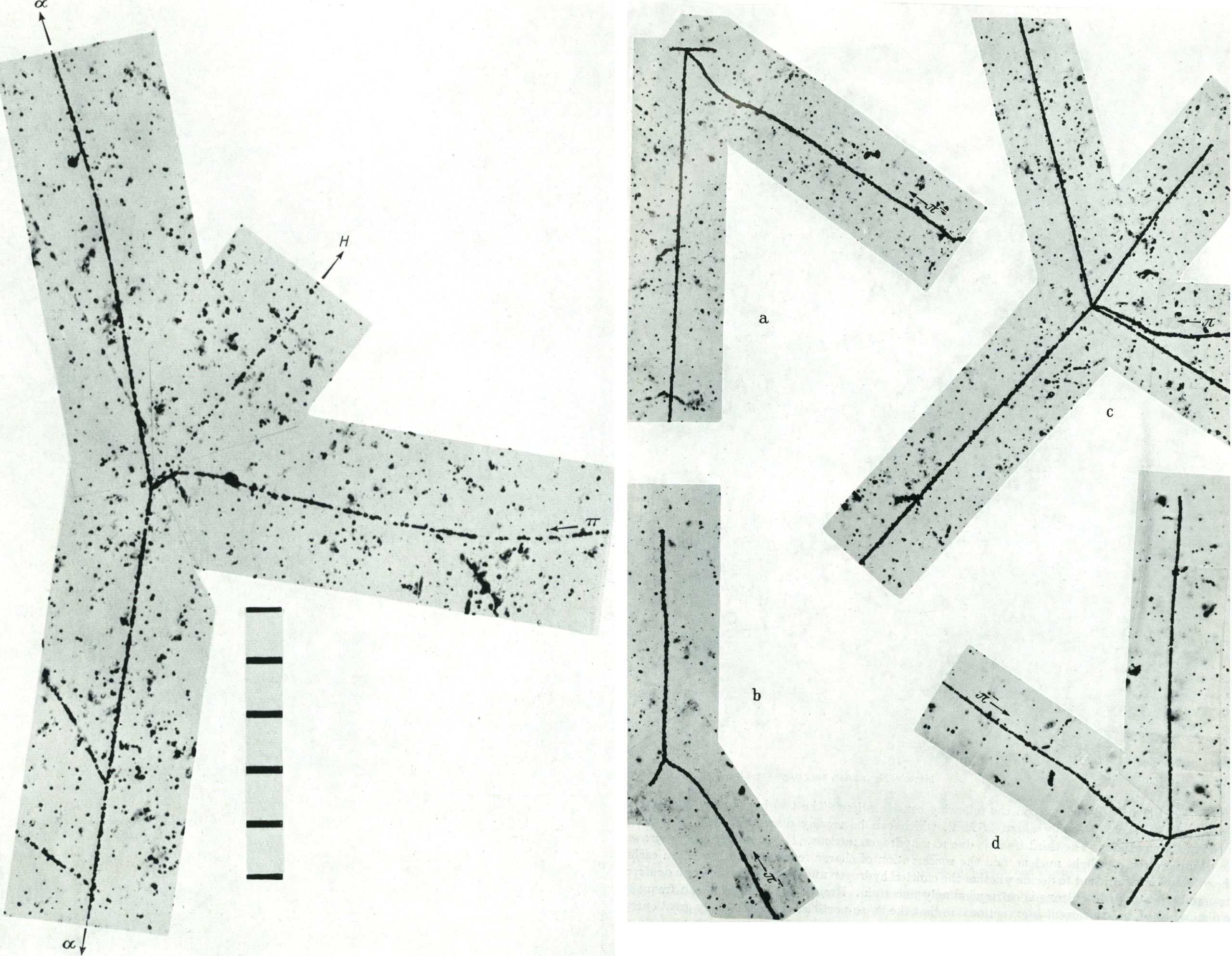
At left, disintegration of a light element, carbon, by the nuclear capture of a π– particle. Reaction of this type can frequently be distinguished, but this event is exceptional in that the two α-particles are or relatively great energy (25 MeV) and are emitted in nearly opposite direction. The emitted hydrogen nucleus was a proton or deuteron (scale is always 50 μm). Pictures at right : Disintegration of light elements, C, N, O by pion capture.
Since the π– particles takes a finite time to be reduced to low velocities when arrested in emulsion, and to cascade down to orbits of low quantum number round the nucleus of the atom by which it is captured, there is a finite probability that it will decay before interacting with a nucleus. This decay is rare, about one in 1000 as said previously.
π production in nuclear collisions
From Powell, 1952 : The first observed production of slow π in nuclear disintegration in photographic emulsion (1947) gave support for the view that the particles interact strongly with nucleons, that they are directly produced in nuclear interactions of great energy, and that, when moving in the atmosphere, they give rise to the weakly interacting muon by decaying ‘in flight’.
The early examples of the creation of pions were all of negative particles. In escaping from a nucleus, the positive particles are commonly accelerated by the electrostatic repulsion. They therefore very rarely appear with low kinetic energy and commonly escape from a thin emulsion before being brought to rest. On the other hand, the negative particles must escape from the parent nucleus against the electrostatic attraction. They are therefore frequently emitted with low velocity, and reach the end of their range in the emulsion.
The apparent origin of pion in nuclear disintegrations, whilst very suggestive, does not prove that the particles are directly created. They might be attributed for example, to decay in flight of a parent neutral particle of mean lifetime less than 10-14 sec. The average distance traveled by a particle with such a lifetime, before decaying, is so short that the track of the pion into which it transformed would appear to originate from the centre of disintegration. That the π are not secondary to such an unstable neutral parent, is strongly suggested however, by the great difference in the frequencies with which slow positive and negative π are observed, an effect which it is difficult to explain in terms of the electrostatic field of the nucleus. Nowadays, we know that the pions are directly created from energy in violent nucleon-nucleon collisions in the upper atmosphere. However, the incoming nucleon, specially proton, must have a minimal kinetic energy to produce pion. For example, in p-p reactions the minimum energy is 280 MeV, below this value the cross-section becomes zero.
Reactions with gamma ray γ production are also possible (p+γ → n+π+ or p + π0) and the minimum energy for γ is Eγ=145 MeV. But this type of photo-reactions are 100 times less probable than inelastic proton-proton collision (cross section is about 120 μbarn for Eγ>500 MeV).
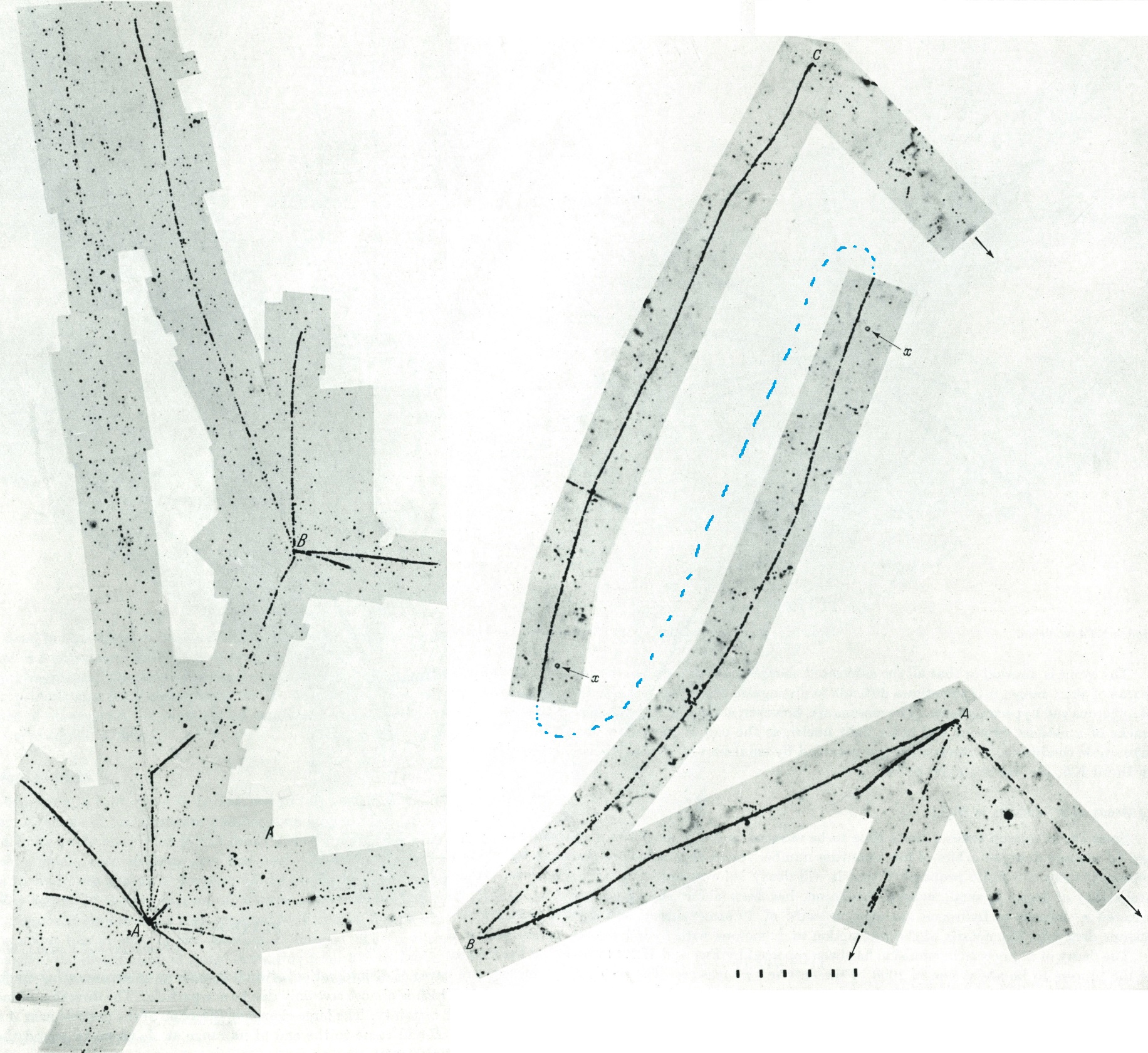
Right : A positive π is ejected from the disintegration at A and decays into a μ at the point B. The track of the μ is given in two-parts (follow the blue dots). The track of the decay electrons, starting from the end of the range of the μ at C, is clearly visible. Left : One of the first two observations of the creation of a π (1947). The π leaves the disintegration at A, and reaches the end of its range at B, where it is captured by a light element and disintegrates it. Two hydrogen nuclei leave the disintegration B moving in an upward direction.
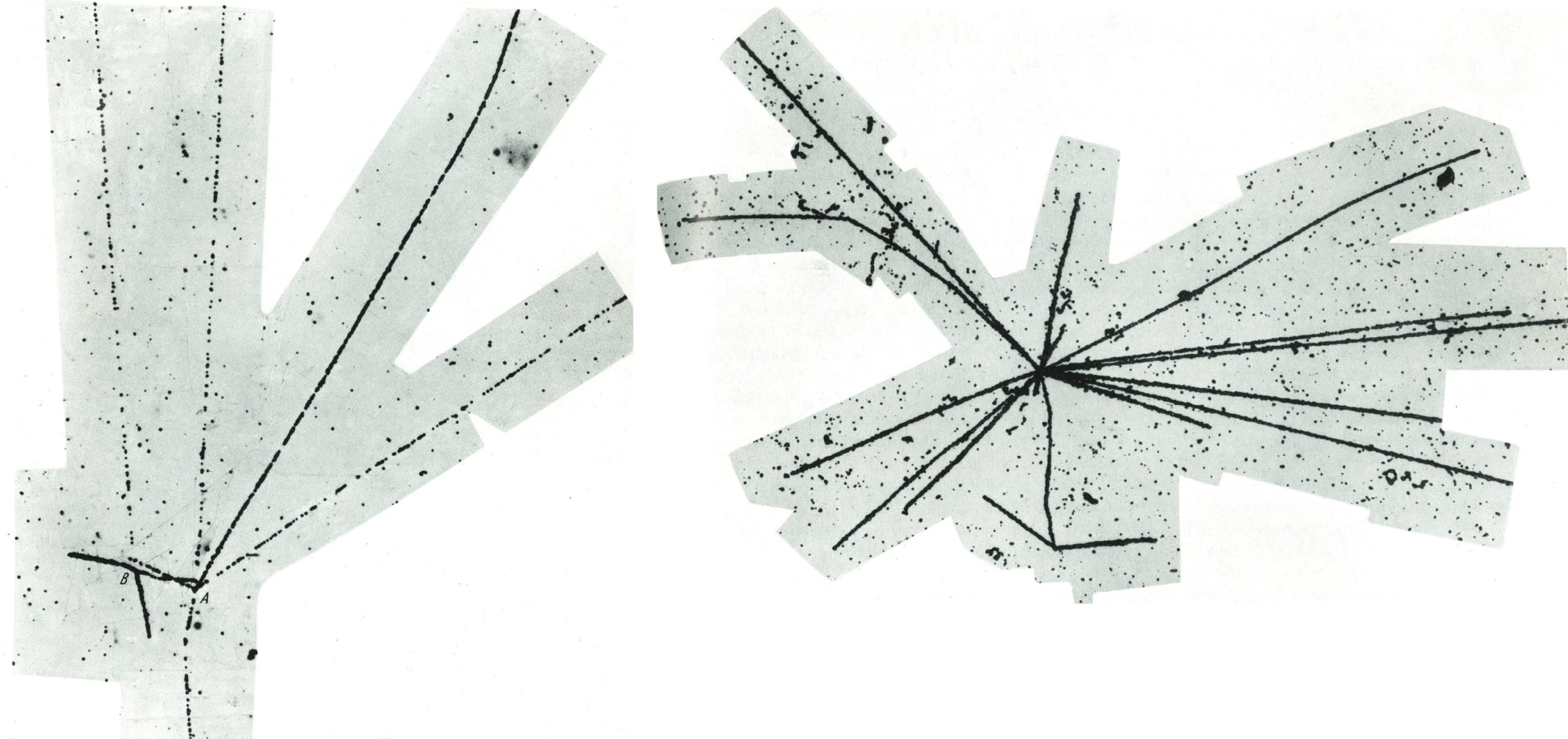
Left : There are two centres of disintegration, A and B, with an interconnecting track, scattered and of high grain-density, which is almost certainly due to a π particle. The direction of motion of the meson (π) cannot be stated with certainty. the large change in direction of the particle near A suggests, however, that it originated at B and came to the end of its range at A, where it caused the disintegration of the nucleus of a light element. Right : The event is unusual in that all the associated charged particles are heavily ionising. In the case of tracks of short range, it is sometimes difficult do distinguish those due to ejected π– from those of hyperons and hyperfragments. The mesons π are, however commonly more scattered.
The neutral π0
Neutral, as well as charged π are emitted in the nuclear disintegrations produced by charged particles of great energy. They commonly decay into two quanta and rarely in an ‘alternative mode’ into a pair of lepton and a γ-ray. Evidence for the production of π0 depend in photographic plates exposed to cosmic radiation depends upon observing the materialization of the γ-rays into which they transform. Occasionally, by chance, the resulting electron-pair is created close to the parent disintegration (left picture). Since the direction of the bisector of the pair points directly to the parent star, the association of the two events is almost certain. In the picture, the disintegration was produced by a neutral particle, probably a neutron. The electron pair are roughly created at 70 μm from the centre of disintegration, this is not the π0 that moved from the centre, but one of the γ-ray from its disintegration because the lifetime of π0 is too short to move in an appreciable distance : Let’s consider that a π0 is emitted at 0,999c : the lorentz factor equal 22. So the π0, and for our referential, the particle have an half life of 22*8,4E-17=1,84E-15 s. This particle can make a distance of 0,55 μm then it will decay, so it’s impossible that a π0 can escape from the centre of disintegration of a nucleus !
Alternative mode of decay π0→ γ +e++e–
It was first pointed out by DALITZ (1951) that general theoretical considerations strongly suggest that the neutral π should also decay in an alternative mode in which one of the γ-rays is replaced by an electron-pair. He estimated that his alternative mode should occur with a frequency about 1% of that of the normal mode. Contemporaneously with the theoretical predictions, CARLSON (1950) observed pairs of electrons to emerge, narrowly inclined to one another, from nuclear disintegrations produced by cosmic radiation. In considering the origin of such electron-pairs, it had first to be excluded that they were not due to the conversion of γ-rays which had emerged from the disintegrating nucleus.
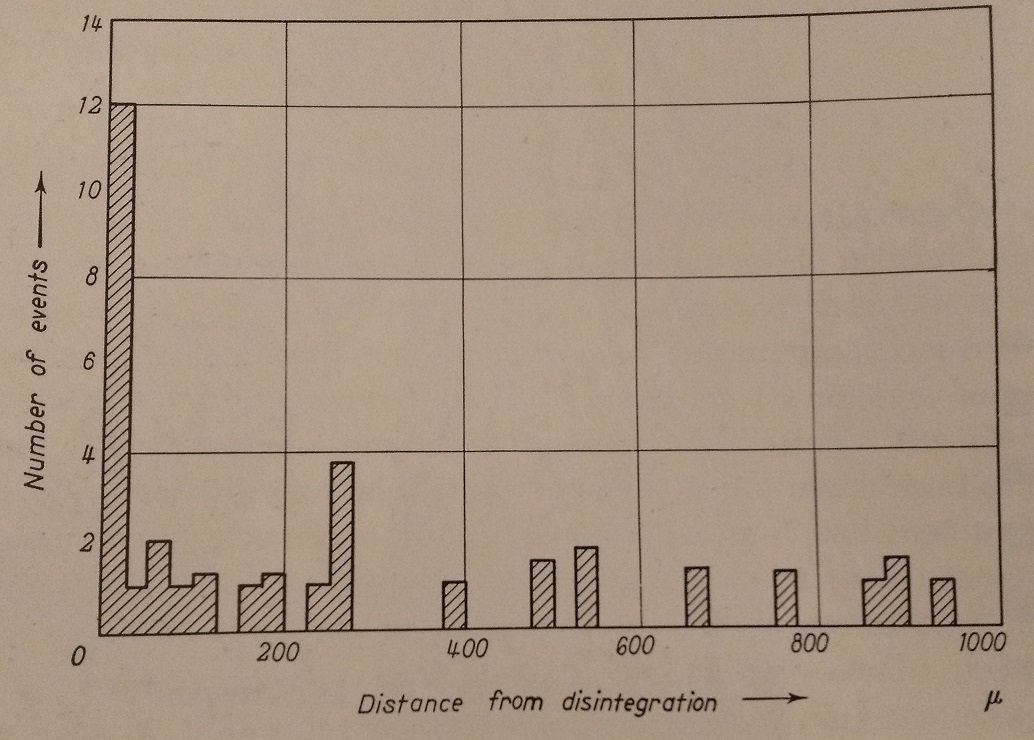
Results from DANIEL. Frequency of occurrence of related electron-pairs as a function of the distance of the point of origin from the parent star. The large number at small distance is due to ‘direct pairs’.
The average length of path that a γ-ray in an emulsion before it materializes into a pair of electrons varies slowly with the quantum energy, but for value of hυ between 10 and 1000 MeV, it’s of the order of 4,5 cm. The chance that a γ-ray, originating in or very near a nuclear disintegration in the emulsion, will produce a pair at a point less that 1 μm from the parent disintegration is therefore only about 0,002%. About sixty such pairs were observed by DANIEL (1952) in conditions in which, if they were due to materialization of γ-rays, only one or two would have been expected (see table in left). Most of the particular pairs could not, therefore, be due to pair production by γ-rays. They are referred to as ‘direct pairs’ and are linked to the alternative mode of decay of the π0. The next picture show the rare alternative mode of decay of π0. In the left-hand photograph, the first grains in the track of the pair cannot be distinguished because of the dense track due to a slow evaporation fragment. The individual electrons were identified by observations of scattering and grain density and their energies are indicated in the photograph. In the right hand photograph only one of the two tracks believed to constitute an electron pair can be identified with certainty.
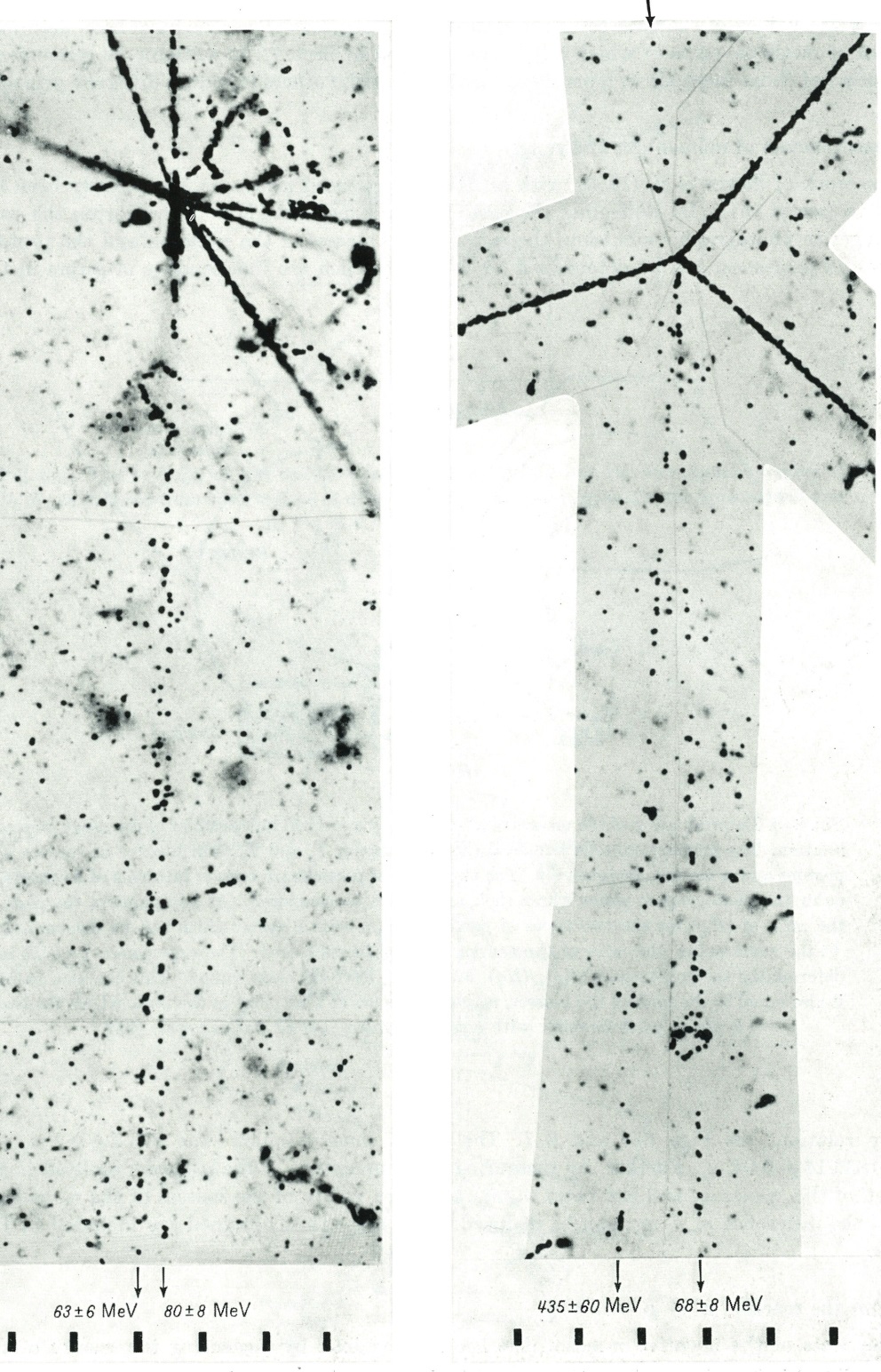
Electron pairs, apparently emerging directly from nuclear disintegrations, attributed to the rare alternative mode of decay of the neutral π meson
s